Physics Achievements of the Fixed Target Area
In the 1970s, uncertainties were the only certainties at Fermilab and in the field of particle physics.
"There were periods of immense confusion," Chris Quigg remembers of those early days in Fermilab's theory group. "Theoretical models were made and discarded every few weeks."
Fermilab's fixed target experiments began clearing the daze. Starting in 1972 the experiments helped, step by step, to establish our present knowledge of the smallest particles and their interactions, the structure of proton, the role of particle interactions in the evolution of the universe. They provided guidance for theorists to find the right description, unearthing the underlying symmetries. Their findings have been essential in creating the Standard Model of particle physics.
The early days
Particle physicists at Fermilab obtained some of their first results operating a fixed target experiment with a 30-inch bubble chamber as their detector. Charged particles leave a track when traveling across a bubble chamber, similar to an airplane contrail leaving a line in the sky.
"Back then the bubble chamber pictures, showing many tracks, were new to everybody and quite striking. [Richard] Feynman carried around the photographs, showing them to everybody," Quigg recalls.
"In 1972, the new accelerator was not yet performing very well. But it was just right for the bubble chamber," says Ernie Malamud, who was among the lab's first 100 employees. "Ten particles were enough for a picture."
Taking tens of thousands of pictures, Fermilab's bubble chamber produced clearer results than any other experiment at that time.
The next-generation detector, a 15-foot bubble chamber, confirmed the existence of neutral current scattering, shortly after Europe's CERN laboratory had recorded the first evidence. Scientists observed that protons sitting in a neutrino beam occasionally received a kick, without any charged particle emerging from the event. Some sort of neutral interaction was taking place. The number of events agreed with the electroweak model, a theoretical framework suggested by Sheldon Glashow, Stephen Weinberg and Abdus Salam, who later won the Nobel Prize. It took another ten years until experimenters discovered the particle mediating the neutral current interaction, the Z boson, at CERN.
The seventies were the heydays of discovering new particles. Many fixed-target experiments aimed at identifying and analyzing hyperons, bound quark states containing at least one strange quark. Similar to Mendeleev's grouping of chemical elements in a systematic table, particle physicists tried to identify the underlying pattern of the many quark bound states they found, a major key in establishing the Standard Model. Fermilab's hyperon experiment E8 made significant contributions. It measured the magnetic moments of hyperons, extensively testing the quark model. Looking at today's particle data tables one finds that many composite particles were discovered, and their properties best measured, at the Tevatron fixed target program.
Dissecting the proton
Using neutrino beams, physicists at Fermilab have studied the structure of the proton at ever-smaller scales. Some of Fermilab's earliest results in this area helped to identify quantum chromodynamics (QCD) as the correct theory of strong interactions, the force combining quarks into little packages. Physicists discovered what is called ëscaling violation,' a specific energy dependence of the neutrino scattering off a quark inside the proton. The simple parton model, viewing the proton as a bag with non-interacting quarks inside, turned out to be wrong. Instead, QCD provided the correct description of the scattering processes, including scaling violation.
Subsequent fixed target investigations at Fermilab, SLAC and CERN have greatly contributed to the understanding of the ëvirtual sea' of quarks and gluons inside the proton. Together with data obtained at Germany's DESY laboratory, where an electron-proton collider is used to study the proton's content, this knowledge of the proton structure is essential for making measurements at the future Large Hadron Collider (LHC) being built at CERN.
Charming events
The simultaneous discovery of the charm quark, the fourth kind of quark, at SLAC's electron-positron collider and a fixed target experiment at Brookhaven in 1974 was a milestone. It led to a wide acceptance of the picture of the quark-lepton substructure of matter. Fermilab, of course, wanted to participate in exploring the properties of charmed particles.
"Even some well-known physicists thought that this kind of physics could only be done with electron-positron colliders, which presumably allow for measurements with much less background," recalls Mike Witherell, former spokesman of E691 and now director of Fermilab. "Not everybody on the program committee was convinced in advance that E691 would work."
Fermilab's set of extremely influential experiments on charm photoproduction, E516, E691 and E791, firmly established the fixed-target program as a first-class source for data on charm quark physics.
"You often find a chain of experiments that over time morphed into some of the most powerful experiments," says experimentalist Chuck Brown, seemingly lifting Fermilab's secret of success.
Fermilab contributed to unraveling the secrets of the production mechanisms behind charmed particles in a fixed target environment, which is completely different from producing them in electron-positron experiments.
"Probably more than half the information we currently have on charm quarks comes from fixed target experiments, yielding beautiful lifetime measurements and branching fractions. The Tevatron fixed target experiments had overwhelming statistical power because they evolved over a long period of time," notes Quigg. "Cornell's electron-positron collider (CESR) and its CLEO detector is another source of high-quality information."
Fermilab's experiment E516 was the first experiment using a full solid angle spectrometer. It recorded particles coming out of a collision and flying off in all directions. Previous experiments used bubble chambers which have limited statistical power because data analysis is slow. Or they used small angle spectrometers, only recording particles flying in certain directions.
"Once you study charm quark physics you have multi-particle interactions," states Tom Nash, a member of E516. "You need to electronically register all particles produced. Then processing this huge amount of data becomes the issue."
E516 was the first experiment to use parallel computing with commercial microprocessors. Computer Farms with more than 50 microprocessors were built.
The follow-up E691 successfully implemented another leap in technology: the use of silicon vertex detectors, which were indispensable encouragement for the future use of hadron beams to do these kinds of measurements.
The discovery of the bottom quark
In 1977, a whole new field of research started at Fermilab with the discovery of the bottom quark, the fifth quark of the standard model. Analyzing data from fixed-target proton-proton collisions, the relative frequency of producing muon-antimuon pairs showed an anomalous peak at about 9.7 GeV. Physicists had discovered the upsilon particle. Further investigation proved this particle to be a bottom quark-antiquark pair.
"Leon Lederman came to Fermilab as the spokesman of E70 and then E288. His proposal for E288 was the shortest I have ever seen," laughs Brown. "It was one page long, and basically said: ëWe hope to discover particles.'"
Instead of the W boson, which Lederman and his collaborators expected to find, they discovered the upsilon.
The bottom quark is today the focus of extensive studies around the world. Its properties may hold the key to many physics questions that cannot be answered in the framework of the standard model.
The matter-antimatter asymmetry
Particle physicists around the world have been able to produce antiparticles for many decades. And they think that a lot of antimatter was present at the beginning of the universe. But where did it all go?
Experiments conducted in the 60s showed that particles and antiparticles do not behave in the same way. There is a slight change in the outcome of some physics processes when replacing all particles by their antiparticles. Physicists speak of CP violation.
So far, measurements of kaon decays offer the only evidence for this matter-antimatter asymmetry. Studying these tiny effects requires very sophisticated fixed-target experiments.
"CERN's NA31/NA48 and Fermilab's E731/KTeV experiments are some of the most beautiful experiments we have in particle physics," Quigg credits his experimental colleagues. "People have evolved the technique over time, built in ways of controlling their systematic errors, understood the details of their analysis."
It is a challenge to measure an effect that is only of the order of one per thousand. Then, measuring this effect to a precision of a tenth of one thousandth is a really big challenge. CERN and Fermilab have achieved this goal, a very important result.
"Theorists will need much more precise calculations to match this," says Quigg realizing that it's the theorists' turn to get as much as possible out of these results. Indeed, the latest experimental results eliminated the possibility of a so-called superweak model to explain the matter abundance in our universe. It is clear, however, that nature must have provided for an extra source of CP violation to create a universe that seems to be made almost entirely of matter, with very little antimatter present.
"We know that CP violation in the standard model of particle interactions is not sufficient to explain the matter excess in our universe. Whether we need some sort of transcription of the standard model or something completely different, we don't know", explains Quigg. "One of the things you hope to find in the next generation of experiments, as you begin to pin things down more, is some systematic deviation from the standard model even for kaon and bottom quark physics."
Answers of the future
The ongoing analysis of data taken during the last phase of the Tevatron fixed target program will hopefully yield yet another first-time observation of a particle. The elusive tau neutrino has never been directly observed. So far it has always been seen indirectly in form of missing energy: An undetected particle carries away some of the total energy entering a collision. The direct observation of the tau neutrino, however, requires identifying the interaction of a tau neutrino with a target nucleus, producing a very short-lived tau particle, a heavy relative of the electron and muon. Physicists of the DoNUT collaboration expect to have recorded enough events. Sifting through the data, however, is not yet complete.
"We know that the standard model is not the final answer," summarizes Quigg. "We want to get to finding the cracks, understanding the electroweak symmetry breaking. The next ten years will be very exciting years for experimental particle physics. A lot of things have the potential to start popping out on us, especially supersymmetry or topcolor. In addition, the wonderful experimental results indicating that neutrinos have a mass and oscillate are tied to the problem of the masses for quarks and leptons. Together with electroweak symmetry breaking, this is the next big problem to be solved."
The commemorative catalog of Fermilab's fixed target program with the Tevatron (1983-2000), edited by Jeff Appel, Chuck Brown, Peter Cooper and Herman White, can be found at http://conferences.fnal.gov/tevft/book/. [This document is available through INSPIRE-HEP]
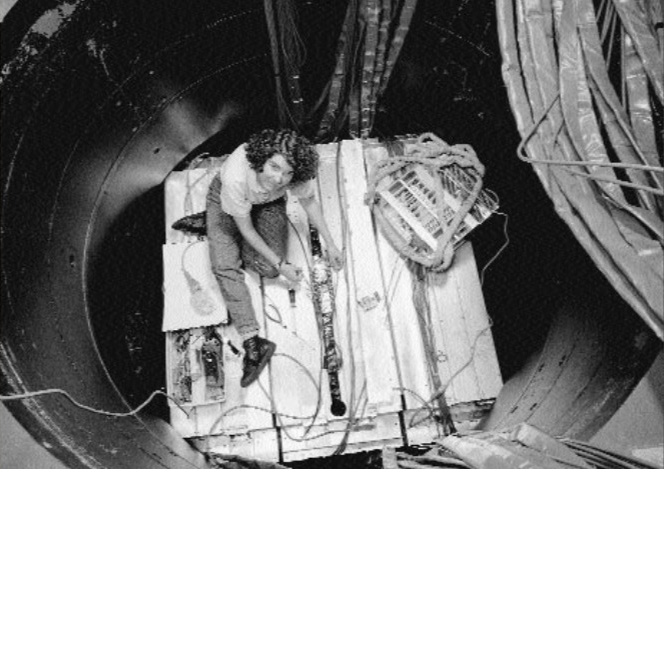
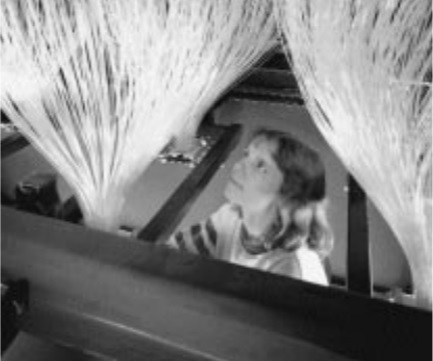